History & evolution
Biosynthesis vs. dietary uptake
DCA and the microbiome
DCA, mitochondria and neurology
DCA and cancer
DCA and immunology
History and evolution
1886: first isolated from ox bile (Hofmann and Hagey 2014) | 1911: first isolated from human samples (Carey and Watson 1955) | 1950s: confirmed as secondary bile acid (Hofmann and Hagey 2014)
Deoxycholic acid (DCA) is a secondary bile acid produced when intestinal bacteria metabolize the primary bile acid cholic acid (Šarenac and Mikov 2018). DCA was first isolated from human fecal samples in 1911 by Fischer, though subsequent investigations focused on cholic acid and rather ignored DCA (Carey and Watson 1955). Interest in DCA was revived in the 1940s, when it was identified as a precursor for the synthesis of corticosteroids (Hofmann and Hagey 2014). Of those, cortisone was a particularly promising new treatment against rheumatoid arthritis, and commercial production boomed after Sarett determined a process for synthesizing cortisone from DCA (Sarett 1946). Since then, other precursors have been used for this application, but DCA still serves in several medical applications, most notably the dissolution of gallstones.
DCA plays an important role in many physiological processes including gut homeostasis, immune cell function and inflammation and is a prominent player in the modulation of bile flow and the absorption of dietary fats. (Su et al. 2023). In research, DCA is used as a detergent to isolate membrane proteins, and is being investigated as a potential component in nanomedicine (Deng and Bae 2023). Because of its ability to disrupt cell membranes in adipocytes and destroy fat cells, DCA is commonly used in lipolysis, either alone or together with phosphatidylcholines, as an injectable to treat “submental fullness” (double chin), though results are mixed (Muskat et al. 2022).
However, bile acids can also have detrimental effects on human health: high exposure to bile acids including DCA seems to be a risk factor for cancer (Ajouz et al. 2014). Current interest in DCA is related to the discovery that bile acids have signaling properties as ligands of receptors in the enterohepatic pathway, meaning that they may be categorized as hormones (Modica et al. 2010). These signaling cascades may help to explain the relationship between diet, gut dysbiosis and complex chronic diseases as discussed below.
Biosynthesis vs. dietary uptake
Cholic acid (CA) and chenodeoxycholic acid (CDCA) are the main primary bile acids formed in humans, derived from cholesterol through a series of hepatic enzymatic reactions (Funabashi et al. 2020). Primary bile acids are conjugated to glycine or taurine to form bile salts that are transported to and stored in the gallbladder. Food consumption prompts the release of bile rich in bile salts from the gallbladder into the small intestine, where they aid the digestion and absorption of lipids, particularly of triglycerides.
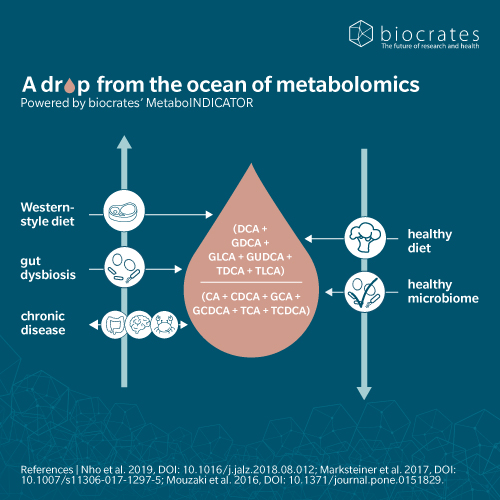
Most primary bile acids are re-absorbed in the distal ileum before entering the colon, but about 5% escape this route and are deconjugated by gut bacteria to serve as substrate fro secondary bile acid synthesis (Ajouz et al. 2014): CA is typically converted to DCA, and CDCA to ursodeoxycholic acid (UDCA) and lithocholic acid (LCA). DCA can also be conjugated with glycine or taurine to form the conjugates GDCA and TDCA, respectively. In humans, DCA is the main secondary bile acid found in the bile acid pool. DCA is mainly re-absorbed in the colon and enters enterohepatic circulation, reaching the liver where it is re-conjugated and secreted in bile.
While this is an endogenous process, it is highly dependent on dietary intake. Fat and fiber consumption influence gut microbiota composition and studies have shown that diet influences DCA levels in the colon (Ghaffarzadegan et al. 2019). Switching to an animal-based diet rich in meat, dairy and eggs led to a 2- to 10-fold increase in fecal DCA levels in human subjects (David et al. 2014). Increasing protein intake on a high fat diet increased plasma DCA by almost 50% (Bortolotti et al. 2009).
DCA and the microbiome
Bile acid concentrations have long been known to influence health and disease. DCA is among the most abundant secondary bile acids, comprising around 20% of the bile acid pool in the bile of adult humans (Hofmann 1999). This amounts to around 500mg, with the excess excreted through feces (Marcus and Heaton 1988). DCA and its derivatives play a vital role in limiting Clostridium difficile production and regulating host metabolism and immune response. High levels of DCA are particularly associated with gut dysbiosis and disease, including hepatocellular carcinogenesis (Funabashi et al. 2020). DCA pool size is also associated with the precipitation of gallstones (Dowling 2000).
A recent study using metabolomic profiling in mice has shown that in addition to the traditional pathways described above, bile acids can be conjugated to amino acids such as phenylalanine, tyrosine, and leucine, by gut microbiota (Quinn et al. 2020). When conjugates and different hydroxyl group states were included, these new “microbially conjugated bile acids” could amount to more than 2800 metabolites, opening up a fascinating new area of research into the impact of bile acid diversity on the wider microbiome (Guzior and Quinn 2021).
The size and diversity of the bile acid pool is regulated by several nuclear and membrane receptors. Bile acids including DCA are ligands of the farnesoid X receptor (FXR), which acts as a “bile acid sensor” to regulate bile acid synthesis (Zhang 2010). Functional FXR is expressed in a wide range of tissues including in brain neurons, the adrenal cortex and pancreatic beta cells. FXR governs the expression of genes related to energy metabolism, bile acid metabolism, insulin signaling, immune cells regulation, mitochondrial physiology, and stability of the tumor suppressor protein p53 (Qiao et al. 2001).
Primary and secondary bile acids have very different actions on FXR. Several primary bile acids are potent agonists, while several secondary bile acids, including DCA, have antagonistic effects (Lefebvre et al. 2009). If the ratios change due to dysbiosis, this can affect the host’s physiology. For example, in non-alcoholic fatty liver disease (NAFLD), bile acid levels are increased but hepatic FXR signaling is downregulated. This is thought to arise from an increase in DCA levels (FXR antagonist) and reduced levels of the primary bile acid CDCA (FXR agonist) (Jiao et al. 2018).
DCA, mitochondria, and neurology
DCA’s emulsifying properties make it very efficient at intercalating within membranes (Sousa et al. 2015). An imbalance in the secondary to primary bile acids ratio can affect cell membranes, especially those already disturbed by high levels of saturated and oxidized fatty acids due, for example to an unhealthy diet. These membranes may become more susceptible to disruption by secondary bile acids, contributing to inflammation, mitochondrial damage, and the production of reactive oxygen species (ROS) and reactive nitrogen species (RNS). Oxidative damage to DNA via ROS and RNS can also promote genomic instability. In addition, DCA can disrupt several DNA mismatch repair enzymes, such as anaphase promoting complex (APC) and p53, by inducing mutations (Liu et al. 2022).
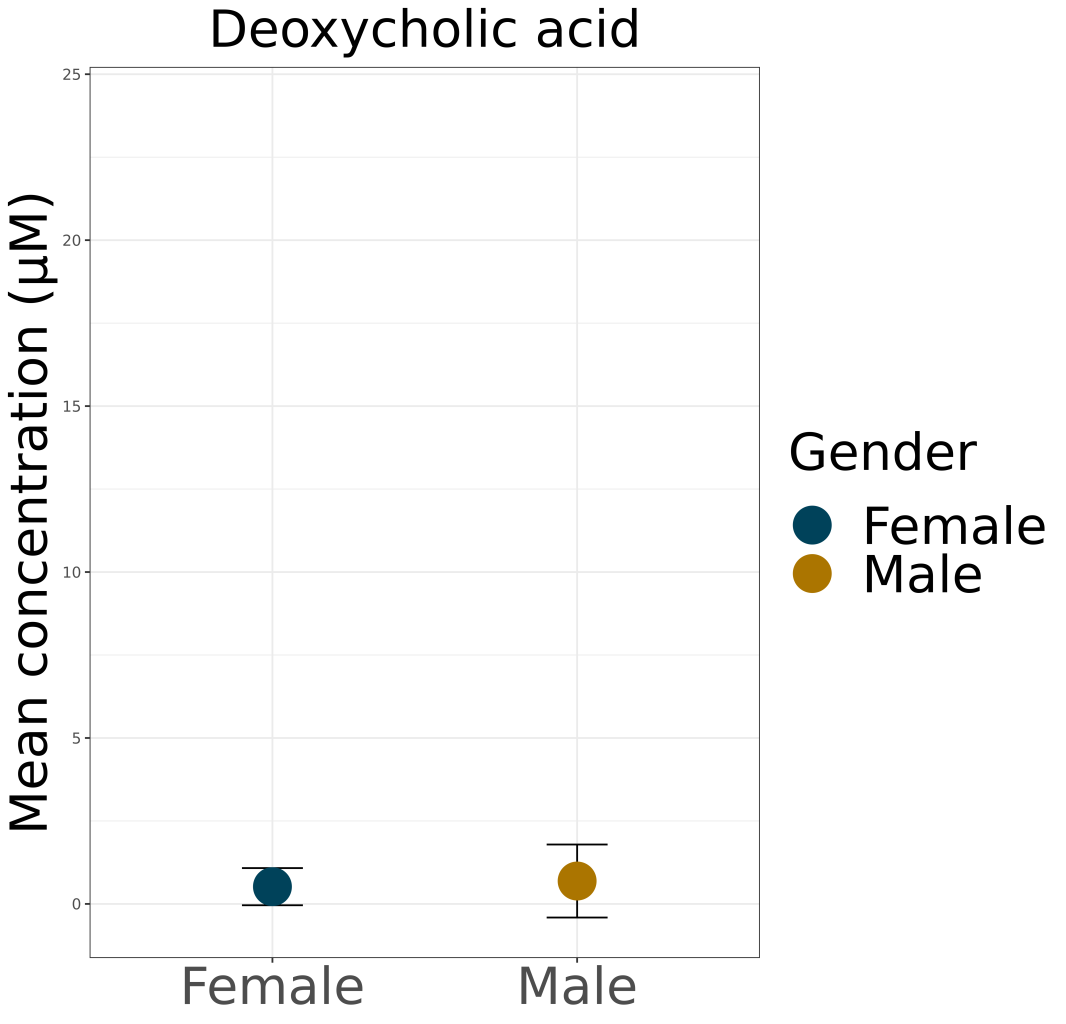
DCA’s effect on mitochondrial metabolism and FXR signaling has implications for the brain. In Alzheimer’s disease (AD), bile acid synthesis has been shown to be affected by FXR. MahmoudianDehkordi et al. (2019) have shown that an increased secondary/primary bile acids ratio in brain tissue and blood correlates with cognitive decline. If high levels of DCA inhibit neuronal FXR, this may lead to other changes in energy homeostasis, such as the down-regulation of glucose transporter-3 (GLUT3) expression, leading to incremental intracellular malnutrition.
When glycolysis cannot be performed due to a lack of intercellular glucose, compensatory processes shift energy supply toward fatty acid oxidation, which increases the demand on the mitochondria. When combined with the membrane disruptions caused by secondary bile acids, this environment can easily lead to mitochondrial dysfunction and incomplete fatty acid oxidation. Such metabolic impairments can be detected in the blood as a complex mixture of acylcarnitines, another marker of late-stage AD (Toledo et al. 2017). This suggests that part of AD pathology may be of metabolic origin and caused by malnutrition in the brain, supporting the reclassification for AD as type 3 diabetes.
DCA and cancer
DCA’s role in tumor growth has been documented since the 1940s (Ajouz et al. 2014). Chronically elevated levels of DCA are associated with colorectal cancer, pancreatic cancer, esophageal cancer and other gastrointestinal cancers. Research suggests that dietary factors play a major role in DCA’s involvement in carcinogenesis, as high levels of bile acids are associated with high fat diets (Ajouz et al. 2014). Population-based studies show similar patterns of elevated secondary bile acid levels in people who consume high-fat and high-beef diets, and in people with colonic carcinomas (Ajouz et al. 2014). A case-control study found a positive association between serum DCA levels and colorectal adenomas (Bayerdörffer et al. 1993).The levels of DCA and other secondary bile acids have often been found increased in the feces of humans and mice fed a high-fat diet, as well as the feces of humans with colorectal cancer ( Liu et al. 2022). Since DCA can promote apoptosis in normal intestinal cells, this could potentially contribute to a carcinogenic environment (Schlottman et al. 2020).
Diet has also been shown to play a role in obesity-related hepatocellular cancers: a mouse model demonstrated that the gut microbiome was altered in obese phenotypes, leading to higher levels of DCA (Yoshimoto et al. 2013). Blocking DCA production and reducing gut bacteria inhibited cancer development in obese mice. Animal studies have also shown DCA to be hepatotoxic (Delzenne et al. 1992). These effects are thought to result from DCA’s involvement in signaling cascades through membrane disruption and receptor activation, as described above.
DCA and immunology
DCA’s role as a signaling molecule is also crucial in the immune response. As well as acting on FXR, DCA acts on G protein-coupled bile acid receptor 1 (GPBAR1 or TGR5), which modulates the inflammatory response (Fiorucci et al. 2018). Both FXR and TGR5 are highly expressed in immune cells. Patients with the autoimmune disease rheumatoid arthritis (RA) have been found to have elevated bile acid levels, linked to proinflammatory cytokine levels, and primary bile acids may be used to predict RA severity (Su et al. 2023). In mice, DCA has been found to enhance macrophage cytokine production via FXR (Yan et al. 2021).
Multiple studies have shown that patients with active inflammatory bowel disease (IBD) have reduced secondary bile acid levels in plasma and stool (Thomas et al. 2022). However, bile acid profiles in early- and late-stage IBD patients vary greatly in response to inflammation-induced microbiome changes, suggesting a correlation between bile acid pools and disease progression. Reports have shown that FXR expression is repressed in early stages of IBD, which again may contribute to changes in microbiome composition and a hyperinflammatory environment.
Learn more about the roles DCA and other secondary bile acids in complex chronic diseases such as cancer, Alzheimer’s disease, depression, inflammatory bowel disease, multiple sclerosis and diabetes in our whitepaper “Complex chronic diseases have a common origin”.
References
Ajouz et al.: Secondary bile acids: an underrecognized cause of colon cancer. (2014) World Journal of Surgical Oncology | https://doi.org/10.1186/1477-7819-12-164
Bayerdörffer et al.: Increased serum deoxycholic acid levels in men with colorectal adenomas. (1993) Gastroenterology | https://doi.org/10.1016/0016-5085(93)90846-5
Bortolotti et al.: High protein intake reduces intrahepatocellular lipid deposition in humans. (2009) The American Journal of Clinical Nutrition | https://doi.org/10.3945/ajcn.2008.27296
Carey and Watson: Isolation of deoxycholic acid from normal human feces. (1955) Journal of Biological Chemistry | https://doi.org/10.1016/S0021-9258(19)81438-2
David et al.: Diet rapidly and reproducibly alters the human gut microbiome. (2014) Nature | https://doi.org/10.1038/nature12820
Delzenne et al.: Comparative hepatotoxicity of cholic acid, deoxycholic acid and lithocholic acid in the rat: in vivo and in vitro studies. (1992) Toxicol Lett | https://doi.org/10.1016/0378-4274(92)90156-e
Deng and Bae: Effect of modification of polystyrene nanoparticles with different bile acids on their oral transport. (2023) Nanomedicine: Nanotechnology, Biology and Medicine | https://doi.org/10.1016/j.nano.2022.102629
Dowling et al.: Review: pathogenesis of gallstones. (2000) Alimentary Pharmacology and Therapeutics | https://doi.org/10.1046/j.1365-2036.2000.014s2039.x
Fiorucci et al.: Bile Acids Activated Receptors Regulate Innate Immunity. (2018) Front Immunol | https://doi.org/10.3389/fimmu.2018.01853
Funabashi et al.: A metabolic pathway for bile acid dehydroxylation by the gut microbiome. (2020) Nature | https://doi.org/10.1038/s41586-020-2396-4
Ghaffarzadegan et al.: Determination of free and conjugated bile acids in serum of Apoe(−/−) mice fed different lingonberry fractions by UHPLC-MS. (2019) Sci Rep | https://doi.org/10.1038/s41598-019-40272-8
Guzior and Quinn: Review: microbial transformations of human bile acids. (2021) Microbiome | https://doi.org/10.1186/s40168-021-01101-1
Hofmann et al.: The Continuing Importance of Bile Acids in Liver and Intestinal Disease. (1999) Arch Intern Med | https://doi.org/10.1001/archinte.159.22.2647
Hofmann and Hagey: Key discoveries in bile acid chemistry and biology and their clinical applications: history of the last eight decades. (2014) J Lipid Res | https://doi.org/10.1194/jlr.R049437
Jiao et al.: Suppressed hepatic bile acid signalling despite elevated production of primary and secondary bile acids in NAFLD. (2018) Gut | https://doi.org/10.1136/gutjnl-2017-314307
Lefebvre et al.: Role of bile acids and bile acid receptors in metabolic regulation. (2009) Physiol Rev | https://doi.org/10.1152/physrev.00010.2008
Liu et al.: Secondary Bile Acids and Tumorigenesis in Colorectal Cancer. (2022) Front Oncol | https://doi.org/10.3389/fonc.2022.813745
MahmoudianDehkordi et al.: Altered bile acid profile associates with cognitive impairment in Alzheimer’s disease – An emerging role for gut microbiome. (2019) Alzheimers Dement | https://doi.org/10.1016/j.jalz.2018.07.217
Marcus and Heaton: Deoxycholic acid and the pathogenesis of gall stones. (1988) Gut | https://doi.org/10.1136/gut.29.4.522
Modica et al.: Deciphering the nuclear bile acid receptor FXR paradigm. (2010) Nucl Recept Signal | https://doi.org/10.1621/nrs.08005
Muskat et al.: The role of fat reducing agents on adipocyte death and adipose tissue inflammation. (2022) Front. Endocrinol | https://doi.org/10.3389/fendo.2022.841889
Qiao et al.: Deoxycholic acid suppresses p53 by stimulating proteasome-mediated p53 protein degradation. (2001) Carcinogenesis | https://doi.org/10.1093/carcin/22.6.957
Quinn et al.: Global Chemical Impact of the Microbiome Includes Novel Bile Acid Conjugations. (2020) Nature | https://doi.org/10.1038/s41586-020-2047-9
Šarenac and Mikov: Bile acid synthesis: From nature to the chemical modification and synthesis and their applications as drugs and nutrients. (2018) Front. Pharmacol | https://doi.org/10.3389/fphar.2018.00939
Sarett et al.: Partial synthesis of pregnene-4-triol-17(beta), 20(beta), 21-dione-3,11 and pregnene-4-diol-17(beta), 21-trione-3,11,20 monoace. (1946) J Biol Chem
Schlottman et al.: Characterization of Bile Salt-Induced Apoptosis in Colon Cancer Cell Lines. (2000) Cancer Res | https://encr.pw/5kwuw
Sousa et al.: Deoxycholic acid modulates cell death signaling through changes in mitochondrial membrane properties. (2015) J Lipid Res | https://doi.org/10.1194/jlr.M062653
Su et al.: Gut microbiota derived bile acid metabolites maintain the homeostasis of gut and systemic immunity. (2023) Front. Immunol | https://doi.org/10.3389/fimmu.2023.1127743
Thomas et al.: The Emerging Role of Bile Acids in the Pathogenesis of Inflammatory Bowel Disease. (2022) Front Immunol | https://doi.org/10.3389/fimmu.2022.829525
Toledo et al.: Metabolic network failures in Alzheimer’s disease: A biochemical road map. (2017) Alzheimer’s & Dementia | https://doi.org/10.1016/j.jalz.2017.01.020
Yan et al.: FXR-Deoxycholic Acid-TNF-α Axis Modulates Acetaminophen-Induced Hepatotoxicity. (2021) Toxicol Sci | https://doi.org/10.1093/toxsci/kfab027
Yoshimoto et al.: Obesity-induced gut microbial metabolite promotes liver cancer through senescence secretome. (2013) Nature | https://doi.org/10.1038/nature12347
Yujing et al.: Secondary Bile Acids and Tumorigenesis in Colorectal Cancer (2020) Frontiers in Oncology | https://www.frontiersin.org/journals/oncology/articles/10.3389/fonc.2022.813745
Zhang et al.: Farnesoid X receptor–Acting through bile acids to treat metabolic disorders. (2010) Drugs Future | https://www.ncbi.nlm.nih.gov/pmc/articles/PMC3899934/